One day in November 2000, developmental biologist Victor Ambros sat down in his office at Dartmouth Medical School to read the latest issue of the journal Nature. Flipping through the pages, he was surprised to see a paper from his friend and sometime collaborator Gary Ruvkun, a molecular biologist at Massachusetts General Hospital.
The paper’s findings jolted Ambros. Seven years earlier, he and Ruvkun had described how a small RNA molecule called a microRNA controls molting in nematodes. Ambros and his team had searched for versions of that microRNA in other species but had come up empty. “I had kind of put the idea aside,” says Ambros, now at the UMass Chan Medical School. But Ruvkun and colleagues had identified a second microRNA and detected it in species across the animal evolutionary tree, as diverse as insects, sea urchins, frogs, and humans. Ruvkun’s results surprised Ambros so much that “I had to set aside 10 minutes to stare out the window and reorganize my view of the universe.”
Ambros isn’t the only person thrown for a loop by discoveries about RNA. Once thought to perform only limited roles in protein synthesis, RNA has proven to be more diverse, capable, and powerful than scientists imagined. “RNA is the central molecule of life,” says John Mattick, an RNA biologist at the University of New South Wales. “Most genes in the human genome specify regulatory RNAs.”
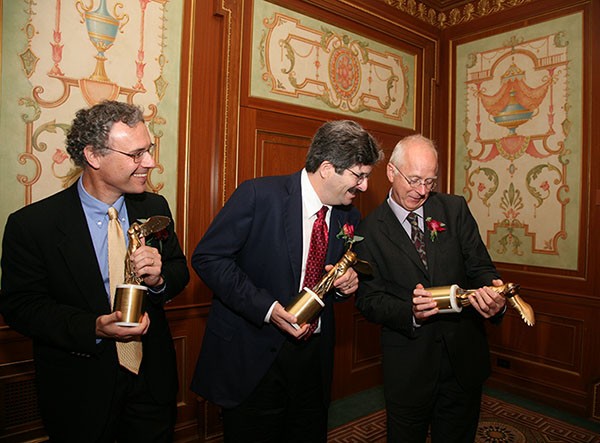
2008 Lasker Basic Award recipients from left to right: Victor Ambros, Gary Ruvkun, and David Baulcombe
Lasker winners helped lead the RNA intellectual revolution. Ambros and Ruvkun shared the 2008 Albert Lasker Basic Medical Research Award with plant scientist David Baulcombe of Cambridge University for uncovering pint-sized RNAs that help manage gene expression and combat pathogens. (In 2024, Ruvkun and Ambros also received the Nobel Prize in physiology or medicine.) Other Lasker winners discovered that RNA can catalyze chemical reactions and revealed that some RNAs go through an unexpected editing process that may have spurred the evolution of eukaryotes—organisms, such as humans, plants, yeast, and fruit flies, whose cells have nuclei. Results from Lasker winners also have spawned approaches for fighting disease. Work by two recipients sped the development of vaccines against COVID-19, saving millions of lives.
On-Time Delivery
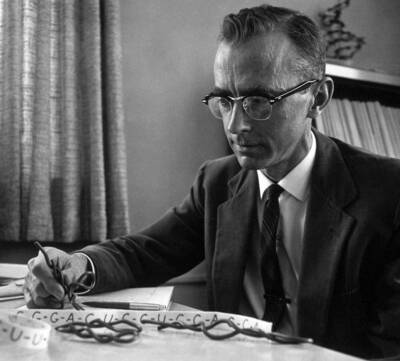
Robert Holley, working for the United States Office of Research and Development during World War II was part of the team at Cornell University that first synthesized penicillin. He won a Lasker Award for determining the chemical structure of an amino acid transfer RNA.
Courtesy of NLM / Science Source
Scientists did not realize until the 1920s that RNA and DNA were distinct types of molecules. By the 1950s, researchers had discovered that certain small RNAs, now known as transfer RNAs (tRNAs), help cells build proteins. As the name suggests, tRNAs specialize in transportation, ferrying amino acids to the site of protein synthesis. Each type of tRNA totes a specific amino acid. In 1958, however, those molecules’ structures were unknown. Robert Holley of Cornell University, who won the 1965 Albert Lasker Basic Medical Research Award, set out to fill that gap.
Holley wanted to figure out the structure of the tRNA that hauls the amino acid alanine. But first he had to get enough of the molecule to work on. Starting with 140 kilograms of yeast, Holley and his team managed to isolate 1 gram of alanine tRNA. But that tiny amount was enough for their experiments. RNA molecules typically consist of strings of four components: adenosine, guanosine, cytidine, and uridine. Holley and colleagues deciphered the order of those building blocks by using enzymes to slice up the tRNA molecule, determining the sequences of the fragments, and then looking for overlaps among the pieces. In 1965, he and his team revealed the full sequence for the tRNA, a scientific first. With that information in hand, the researchers could deduce the tRNA’s structure and begin to investigate other questions, such as how the molecule interacts with other RNAs during protein synthesis. For the work, Holley also shared the 1968 Nobel Prize in physiology or medicine.
Going Through the Process
Although cells make RNAs in the nucleus, the molecules do some of their best work in the cytoplasm. James Darnell of the Rockefeller University earned the 2002 Lasker Special Achievement Award in Medical Science in part for determining what happens to RNA molecules between those locations. In the early 1960s, Darnell began following newly made RNAs and noticed that the nucleus harbored some enormous specimens of the molecules. Darnell and colleagues later determined that those RNAs became parts of ribosomes, the cell’s protein-making organelles. Cells were trimming the precursors of ribosomal RNAs before dispatching them to the cytoplasm.
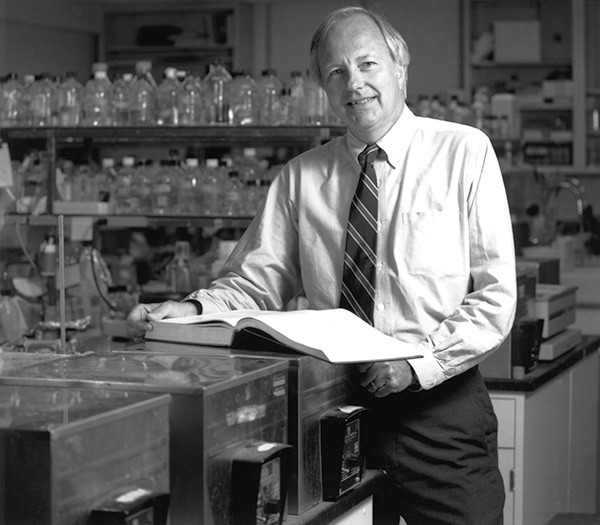
James Darnell in his lab at Rockefeller University in 1989
Courtesy of Rockefeller University
Darnell and Klaus Scherrer identified another type of hefty RNA molecule in the nucleus that they suspected was the forerunner of messenger RNA (mRNA), the RNA variety that contains the instructions for making proteins. Darnell, Scherrer, and other researchers added credence to that hypothesis by determining that the oversized RNA picks up a long tail of adenine molecules. mRNA also sports that type of tail. However, researchers didn’t understand how an mRNA precursor molecule, or pre-mRNA, got smaller during its journey to the cytoplasm.
Genes, Interrupted
Phillip Sharp of MIT solved that problem, making a discovery that Mattick dubbed the biggest surprise in molecular biology. Sharp found that as they prepare pre-mRNAs for use in protein synthesis, cells remove sequences called introns from the molecules. A bit like outtakes from a movie, introns don’t code for portions of proteins. Another team, led by molecular biologist Richard Roberts of the Cold Spring Harbor Laboratory, simultaneously came to the same conclusion. Uncovering that editing process, known as splicing, earned Sharp the 1988 Albert Lasker Basic Medical Research Award. He split the 1993 Nobel Prize in physiology or medicine with Roberts.
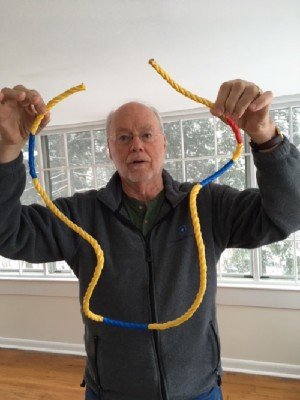
Phillip Sharp explains the concept of RNA splicing with rope colored to represent introns and exons.
An mRNA molecule is the working copy of a gene’s DNA. Ribosomes read the mRNA and build its specified chain of amino acids. In 1977 researchers assumed, mainly from studies of bacteria, that a gene’s nucleotide sequence corresponds to the sequence of amino acids in its protein. As far as scientists knew, genes had no superfluous sequences.
Sharp and colleagues found otherwise when they infected mammal cells with a virus whose DNA genome slips into the nucleus. Once that happens, the cell begins to fashion viral mRNAs. Sharp’s team mixed fragments of viral mRNA with the corresponding sections of viral DNA. Because the DNA and RNA molecules were complementary, the fragments should have stuck together seamlessly. Instead, sections of the DNA protruded, forming loops indicating that sequences in the virus’s genome were missing from the mRNA. Sharp and colleagues proposed that the cells had edited out those segments during the formation of mRNA.
“Nobody in their right mind expected genes to be fragmented,” Mattick says. But further research quickly confirmed that introns are sprinkled throughout eukaryotic genes. After clipping the introns out of pre-mRNAs, cells stitch together the remaining sequences to produce mRNAs. The process probably is advantageous because of the flexibility it confers. Organisms can create various proteins by splicing their mRNAs at different positions. In turn, that versatility probably helped eukaryotes evolve into a huge range of forms, says biochemist Gerald Joyce of the Salk Institute for Biological Studies. “Splicing is the ‘complexifier’ of eukaryotes.”
Making Sense of snRNPs
RNAs undergo splicing, but they also help perform it. Molecular biologist Joan Steitz of Yale University earned the 2018 Lasker~Koshland Special Achievement Award in Medical Science in part for her work to uncover certain RNAs’ roles in splicing.
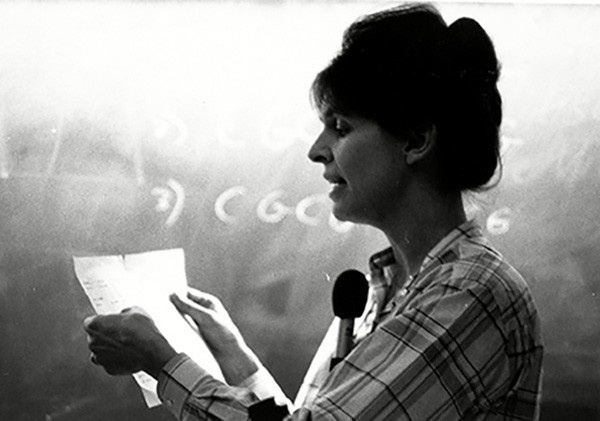
Joan Steitz presents at the Cold Spring Harbor Symposium on Quantitative Biology Structures of DNA, 1982.
Courtesy of Cold Spring Harbor Laboratory Archives
Steitz already had distinguished herself in RNA research for a study she performed in the late 1960s showing how ribosomes recognize mRNA. Ribosomes begin reading the mRNA at a specific site, but how the organelles determined where to latch on was not clear. Using a clever experiment, Steitz identified a “start here” sequence in mRNA that attracted the organelles.
By the late 1970s, she had turned her attention to splicing, whose mechanism remained murky. Steitz and her team wanted to determine whether particles in the nucleus called small nuclear ribonucleoproteins (snRNPs) took part in splicing. But snRNPs, which contain RNA and proteins, were hard to isolate until the researchers found that antibodies from lupus patients glom onto the particles. After nabbing snRNPs, the researchers could probe the particles’ functions. Steitz and colleagues helped show that snRNPs perform key jobs during splicing. In 1983, for instance, her group showed that one snRNP identifies splicing sites on the pre-mRNA. Researchers now know that snRNPs converge to form a larger splicing structure called the spliceosome that contains five kinds of small RNA molecules and about 100 proteins.
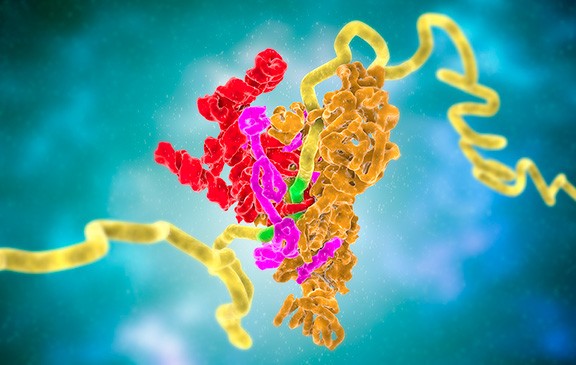
Molecular model of a spliceosome: pre-messenger RNA (yellow) pairs complementary sequence of its splice site (green). Following this binding, additional protein (orange, pink) and RNA subunits are recruited to assemble the rest of the spliceosome complex.
Courtesy of RAMON ANDRADE 3DCIENCIA / Science Source
Life Before DNA?
Splicing, coding for proteins, and transporting amino acids are just three of RNA’s many talents. An experiment that appeared to go awry led to a bombshell discovery about the molecule’s abilities. In the early 1980s, Thomas Cech of the University of Colorado and Sidney Altman of Yale University found that some RNA molecules could catalyze chemical reactions by acting as enzymes. Researchers had thought that only proteins could do that. For that work, Cech won the 1988 Albert Lasker Basic Medical Research Award and the 1989 Nobel Prize in chemistry shared with Altman.
Cech and colleagues were studying how cells make the RNA for ribosomes. Having noticed that the RNA contained an intron, the researchers decided to isolate the enzyme cutting it out. To some test tubes, Cech’s lab technician, Arthur Zaug, added ribosomal RNA and the contents of cell nuclei, which the researchers assumed would contain the splicing enzyme. Zaug also set up control tubes that lacked the nuclear material. Analyzing the results, the pair found that the intron had been spliced out in both the experimental and in control tubes. “Well, Art, this looks very encouraging, except you must have made some mistake making up the control sample,” Cech told his technician. But when Zaug repeated the procedure, the results were the same.
Through a series of what Mattick calls really careful experiments, Cech and his team showed that RNA molecules could serve as catalysts. Even more surprising, the RNA acted on itself. At the time, researchers still thought that RNA’s work was limited to protein synthesis, but Cech’s findings showed that “RNA is not a passive information carrier. It can do things,” Joyce says. Scientists have since discovered other RNA catalysts, such as the RNAs in ribosomes and the spliceosome.
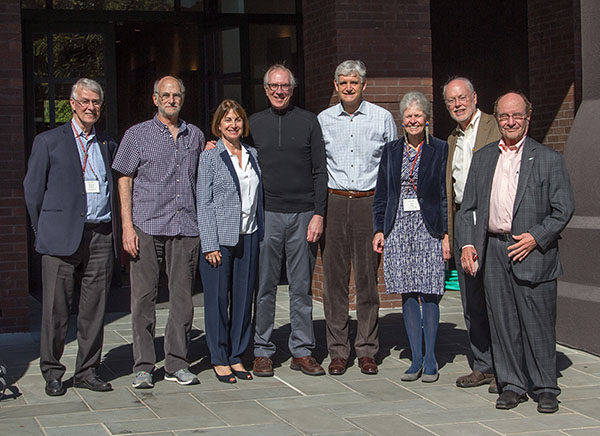
2007 History of mRNA Splicing meeting in Cold Spring Harbor with from left to right: Rich Roberts, Michael Rosbash, Mila Pollock, Thomas Cech, Bruce Stillman, Joan Steitz, Phillip Sharp, and Walter Gilbert
Courtesy of Cold Spring Harbor Laboratory Archives, NY
The results also bolstered a hypothesis about life’s origin. Explaining how DNA could arise without proteins, or vice versa, was a conundrum for researchers trying to understand how life got started. In the late 1960s, researchers posited that RNA was the first enzyme, but they had no direct evidence for the hypothesis. By confirming that RNA molecules can catalyze reactions, Cech’s findings supported the idea. Scientists surmised that an RNA world, in which RNA took on the tasks of DNA and proteins, might have preceded the DNA-based life we know today. That hypothesis remains the leading explanation for how life began.
Small RNAs With a Big Impact
Cech’s work expanded scientists’ understanding of RNA’s repertoire. Research by Ruvkun, Ambros, and Baulcombe uncovered yet more unexpected functions for the molecule in controlling gene expression and defending against pathogens.
Ruvkun and Ambros met in the early 1980s as postdoctoral researchers in the same MIT lab. They clicked, Ruvkun says. “He is smart as can be and nice as can be, and that’s a good combination for working together.” The pair wanted to understand how genes shape development of nematodes and had zeroed in on two genes that, when mutated, led to abnormalities. Worms with certain mutations in one gene, lin-4, grow to adult size, but some organs don’t form. By contrast, worms with particular glitches in the other gene, lin-14, mature early but are puny. Working together, the pair discovered that lin-4 blocked lin-14, but they didn’t know how.
When the two scientists left MIT for faculty jobs at different institutions, they divvied up the work. Ruvkun took lin-14 and Ambros took lin-4. Over the next several years, Ruvkun and his team determined lin-14’s sequence and found that it serves as a master gene that controls the timing of key changes during worm development. Then it was Ambros’s turn to astonish Ruvkun. Both scientists expected that lin-4 would code for a protein. But Ambros’s lab discovered that the gene instead encoded a dainty RNA only 22 nucleotides long. The revelation changed everything, Ruvkun says.
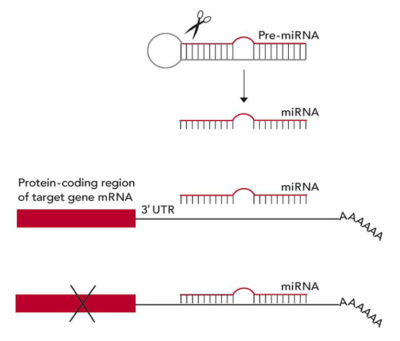
This scheme shows how one type of tiny RNA, a microRNA (miRNA), silences genes. It is cut out of a precursor hairpin-shaped pre-miRNA to form a mature miRNA, which binds to the 3′ untranslated region (3′ UTR) of a target gene’s messenger RNA and turns off its activity.
In 1992, the pair swapped the sequences of lin-4 and lin-14 and shared their conclusions during what Ruvkun says was a mythic phone call. They realized that the sequence of lin-4 partially matched the sequence of a region of lin-14. Their experiments indicated that the lin-4 RNA dials back production of the lin-14 protein by targeting that region in lin-14’s mRNA. “The mechanism was obvious and kind of intuitive right off,” Ambros says. lin-4 was the first discovered microRNA.
Other researchers mostly yawned, however, because lin-4 appeared to be a quirk of nematodes. But in 2000 Ruvkun and colleagues discovered the second microRNA and showed that it was widespread in animals, pointing to what he describes as an unsuspected world of tiny RNAs. Other studies began to uncover scores of the molecules. “That’s when people realized that their favorite gene could be regulated by a microRNA,” says molecular biologist David Bartel of MIT, who wasn’t involved with Ruvkun’s or Ambros’s work. Researchers have since identified thousands of microRNAs, including more than 500 in humans. MicroRNAs tamp down gene expression in various genetic pathways in almost all organisms. And evidence suggests that microRNAs regulate the mRNAs from most human genes. The molecules are important not only for directing development but also for controlling cell functions in adults, and research has implicated them in an array of diseases, including cancer.
Baulcombe discovered a parallel system that also involves small RNAs. His lab was essentially vaccinating plants against viral diseases. The researchers inserted viral DNA into the plants’ cells, stimulating them to make viral mRNA, which prompted a protective response. “I’ve always looked for stuff that doesn’t make sense,” Baulcombe says, and he focused on an incongruous result from the experiment. The plants with the highest resistance carried very little of the viral mRNA, whereas vulnerable plants harbored an abundance of the molecule. The resistant plants appeared to have turned down production of the mRNA.
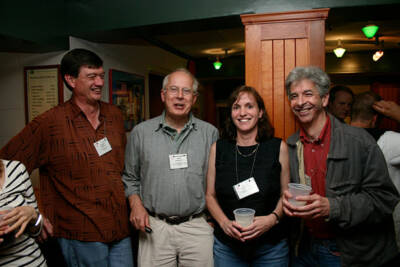
2006 Symposium on Regulatory RNAs with from left to right: Richard Jorgensen, David Baulcombe, Marja Timmermans, and Ron Plaster
Courtesy of Cold Spring Harbor Laboratory Archives, NY
Baulcombe wondered whether a small RNA molecule was silencing the viral mRNA. He and colleagues began hunting for such a molecule. They injected tomato plants with snippets of viral DNA and then used a technique called gel electrophoresis to separate RNA molecules from samples of the plants’ tissues. But the putative small RNA proved elusive until one day in the late 1990s, when Baulcombe’s postdoctoral student, Andrew Hamilton, decided to leave work early to play soccer. When Hamilton cut the gel electrophoresis procedure short, the researchers saw the first evidence of an RNA with a mere 25 nucleotides. The scientists had discovered small interfering RNAs, or siRNAs.
siRNAs differ structurally from microRNAs and function slightly differently. But both are minute and regulate genes by homing in on mRNAs. Small RNAs’ first job was probably defense, Baulcombe says. For that function, “RNA silencing is absolutely brilliant” because it is “targeted against the viral genome” and spares the host’s genome. Organisms then probably co-opted small RNAs for gene regulation, he says.
Researchers didn’t expect small RNAs to be so powerful, and one reason that Baulcombe, Ruvkun, and Ambros could uncover the molecule’s functions is that they were open-minded about their findings, Bartel says. “That was critical to their continuing to work on this, even when their observations could not easily be explained.”
An RNA Shot in the Arm
The speed with which researchers developed mRNA-based vaccines against SARS-CoV-2 is a prime illustration of the improved understanding of RNA. Immunologist Drew Weissman of the University of Pennsylvania and biochemist Katalin Karikó, then with the biotech firm BioNTech, shared the 2021 Lasker~DeBakey Clinical Medical Research Award and the 2023 Nobel Prize in physiology or medicine for discoveries that accelerated that process. The pair “put the pieces together” to allow creating vaccines in record time, says Paul Duprex, a molecular virologist at the University of Pittsburgh who wasn’t involved with Weissman and Karikó’s work.
For decades, vaccine development had followed pretty much the same path, Duprex says. Researchers isolated the pathogen responsible for a disease and then weakened it, either by growing it for many generations in nonhuman cells or by treating it with chemicals. For example, Jonas Salk, who won the 1956 Albert Lasker Clinical Medical Research Award for producing the first polio vaccine, used formaldehyde to inactivate the poliovirus without compromising its ability to rouse the immune system. Once a pathogen was tamed, vaccination could begin. Although researchers have introduced some twists to the process, vaccine development stayed slow, taking years or even decades.
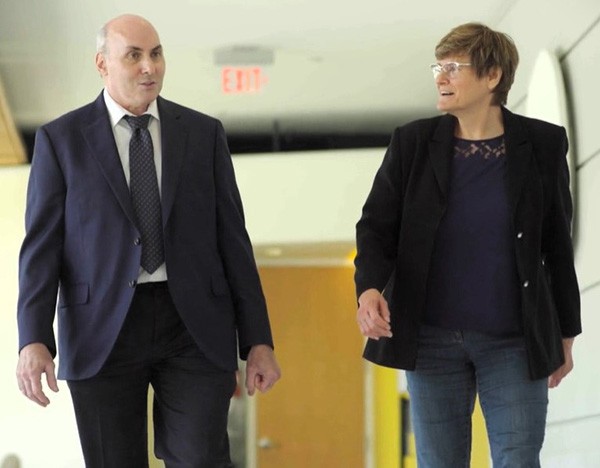
Drew Weissman and Katalin Karikó in 2022
Courtesy of Thomas Media
More than 30 years ago, researchers proposed a different strategy that involved injecting an mRNA molecule that coded for a viral protein. Cells would then make the viral protein, spurring the immune system to produce defenses against the virus. The procedure could be faster because researchers wouldn’t have to grow the pathogen. They could use the sequence of the virus’s genome to quickly make the necessary mRNA.
But for plenty of reasons, an mRNA vaccine might not work. RNA breaks down rapidly in the body, so an injected mRNA might not reach target cells. Moreover, unfamiliar RNA can signal a viral infection, and an mRNA vaccine could unleash dangerous inflammation.
Karikó and Weissman, who began working together in the late 1990s, overcame several obstacles to mRNA vaccination. To prevent the vaccine from provoking inflammation, the duo took a cue from tRNAs, which swap out some of their uridine molecules for a related molecule called pseudouridine. The difference between the molecules is very small—“next to nothing,” Duprex says. But it makes pseudouridine less provocative to the human immune system. In 2005, Weissman and Karikó reported that replacing some uridine molecules with pseudouridine allowed mRNAs to dodge immune cell recognition. Weissman’s lab also showed in 2015 that tiny molecular containers called lipid nanoparticles serve as excellent carriers for mRNA molecules. When the researchers injected nanoparticles containing mRNAs into mice, the rodents’ cells took up the mRNAs and began making the proteins they encoded.
By the time SARS-CoV-2 began spreading in late 2019, several mRNA-based vaccines for diseases such as flu had already reached clinical trials. Drug companies could thus quickly pivot to making vaccines against the new virus. In 2024, the Food and Drug Administration approved the first mRNA vaccine for an illness other than COVID-19. The vaccine targets infections with respiratory syncytial virus. Researchers are studying whether mRNA vaccines can fight other pathogens, including HIV, Zika virus, and norovirus, as well as diseases such as cancer.
The findings from Lasker winners who probed RNA helped revamp the molecule’s image. Partly because of their work, RNA is at the center of studies to understand the origin of life, the organization of the genome, the control of development in multicellular organisms, and the function of the brain. The molecule also is the focus of research to develop approaches to prevent and fight disease. Once dismissed as DNA’s gofer, RNA has definitely come up in the world.
By Mitchell Leslie